Topic Menu
► Topic MenuTopic Editors



Antennas
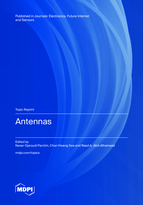
A printed edition is available here.
Topic Information
Dear Colleagues,
Due to rapid growth in the area of modern wireless communication systems, the demand for different types of novel, multi-functional, and high-performance antennas is increasing exponentially. As a crucial part of the future communication system, breakthrough in the development of antennas will obviously improve the performance of the whole communication system. Over recent years, considerable research efforts have been directed toward the development of antennas. The adaptation of the antenna design and technologies for various wireless services requires careful consideration to meet demanding specifications. Having wide and multiple frequency coverage, compact size, well-defined radiation coverage, multi-mode operation, low-cost and ease of fabrication, energy-efficiency, ease of integration and assembly, and conformity are some examples of the key parameters that ensure the success of antenna systems for current and future wireless communication systems. In addition, MIMO and phased array arrangements of antenna systems with multiple adaptive and smart antennas can significantly enhance the system capacity toward meeting the requirements of future wireless networks. Therefore, advanced antenna design techniques that use novel approaches and address various aspects are required.
The objective of this Topic is to cover all aspects of antennas used in existing or future wireless communication systems. The aim is to highlight recent advances, current trends, and possible future developments of antennas. To further promote the development of this area, we invite researchers to submit their original research or review papers that are concerned with novel design techniques, analysis, signal processing, optimization, prototyping, and experimentation in this area.
Submissions can focus on conceptual and applied research in subjects including but not limited to the following:
- Antenna Design
- RFID Tag Antennas
- 4G/5G/6G Antennas
- Antenna Calibration
- Integrated Antennas
- Smartphone Antennas
- Antenna Optimization
- Antenna Miniaturization
- Filtering Antenna Design
- Reconfigurable Antennas
- Beam Forming Techniques
- Mutual Coupling Reduction
- Energy Harvesting Antennas
- Antenna Feeding Techniques
- Distributed Antenna Systems
- Adaptive and Smart Antennas
- Electromagnetic Bandgap (EBG)
- UWB and Multi-Band Antennas
- Antenna Array Signal Processing
- Diversity Techniques in Antennas
- Reconfigurable Intelligent Surfaces
- Decoupling Techniques of Antennas
- In Situ Characterization of Antennas
- MIMO and Massive MIMO Antennas
- Antennas for Biomedical and WBAN
- MM-Wave, THz, and Nano Antennas
- Substrate-Integrated Waveguides (SIW)
- Transmission and Detection Techniques
- Textile, Wearable, and Flexible Antennas
- Prototyping and Manufacturing Methods
- Metasurfaces and Reflect Array Antennas
- Phased Array and Beamforming Antennas
- Automotive, Radar, and Satellite Antennas
- Antenna Design for Massive MIMO and IoT
- Dual-Polarized/Circular-Polarized Antennas
- Angle of Arrival Estimation Using Antennas
- Artificial Intelligence (AI) Empowered Antennas
- Measurements and Experimentation of Antennas
- Channel Capacity Estimation of Antenna Systems
- Antenna on Chip (AoC) and Antenna in Package (AiP)
- Advanced Algorithms of Array Analysis and Synthesis
- Antennas for Complex Radio Wave Propagations Scenarios
- Advanced Techniques for Numerical Modelling of Antennas.
Submissions should reflect the high quality of this international journal and should not have been submitted or published elsewhere. Extended versions of conference papers that show significant improvement (minimal of over 50%) can be considered for publication in this Topic.
Dr. Naser Ojaroudi Parchin
Dr. Chan Hwang See
Prof. Dr. Raed A. Abd-Alhameed
Topic Editors
Participating Journals
Journal Name | Impact Factor | CiteScore | Launched Year | First Decision (median) | APC |
---|---|---|---|---|---|
![]()
Electronics
|
2.6 | 5.3 | 2012 | 16.8 Days | CHF 2400 |
![]()
Future Internet
|
2.8 | 7.1 | 2009 | 13.1 Days | CHF 1600 |
![]()
Sensors
|
3.4 | 7.3 | 2001 | 16.8 Days | CHF 2600 |
![]()
Telecom
|
2.1 | 4.8 | 2020 | 22.7 Days | CHF 1200 |
MDPI Topics is cooperating with Preprints.org and has built a direct connection between MDPI journals and Preprints.org. Authors are encouraged to enjoy the benefits by posting a preprint at Preprints.org prior to publication:
- Immediately share your ideas ahead of publication and establish your research priority;
- Protect your idea from being stolen with this time-stamped preprint article;
- Enhance the exposure and impact of your research;
- Receive feedback from your peers in advance;
- Have it indexed in Web of Science (Preprint Citation Index), Google Scholar, Crossref, SHARE, PrePubMed, Scilit and Europe PMC.