ABSTRACT
Classical novae, explosions that result from thermonuclear runaways (TNRs) on the surfaces of white dwarfs (WDs) accreting hydrogen‐rich matter in close binary systems, are sporadically injecting material processed by explosive hydrogen‐burning nucleosynthesis into the interstellar medium (ISM). Although novae probably have processed less than ∼0.3% of the interstellar matter in the Galaxy, both theoretical and observational evidence suggests that they may be important sources of the nuclides 7Li, 15N, and 17O, as well as the radioactive isotopes 22Na and 26Al. The latter nuclides are astrophysically important in that they may have been involved in the production of the 22Ne (Ne‐E) and 26Mg enrichments identified in meteoritic inclusions, the composition of which is thought to be representative of the chemical and mineral contents of the primitive solar nebula. These inclusions may be partially composed of dust condensed in nova outbursts. We review theoretical expectations for the yields of various isotopes in nova outbursts and conclude that any of the heavy isotope anomalies attributable to novae are most likely produced by the approximately 25%–33% of novae that occur in systems containing massive (M*>1.2 M⊙) oxygen‐neon‐magnesium (ONeMg) WDs. We attempt to place quantitative constraints on the degree to which classical novae participate in the production of chemical anomalies, both in the primitive solar system and on a Galactic scale. Diffuse Galactic γ‐ray fluxes provide particularly important clues to and constraints on the 22Na and 26Al yields from novae. Ultraviolet (UV), optical, and infrared (IR) emission‐line spectra of classical novae reveal the abundances of some of the gas‐phase elements present in the ejecta; recent results are reviewed. We describe how IR observations of novae reveal dust formation and gas‐phase line emission and how they distinguish the temporal development of nova explosions on carbon‐oxygen (CO) WDs (CO novae) from those on ONeMg WDs (ONeMg or "neon" novae). Recent studies show that the ejecta in some novae can be strongly cooled by near‐ and mid‐IR forbidden‐line radiation from highly ionized ("coronal") atomic states. We compare the abundances deduced from recent UV, optical, and IR observations with theoretical predictions, and we suggest that future studies of IR coronal emission lines may provide additional key information. Novae produce only about 0.1% of the Galactic "stardust" (dust condensed in stellar outflows), but IR observations show that it may be some of the more interesting dust. Novae appear capable of producing astrophysical dust of virtually every known chemical and mineral composition. We summarize recent IR observations of the dust production scenario in novae and argue that neon novae may lead to the formation of dust grains that carry the Ne‐E and 26Mg anomalies.
Export citation and abstract BibTeX RIS
1. INTRODUCTION
Observations of Galactic classical novae show that they participate in a cycle of Galactic chemical evolution in which grains and gas in the ejecta of evolved stars are a significant reservoir for the transportation of metals in the Galactic ecosystem. During the late stages of stellar evolution, metals produced during post–main‐sequence nucleosynthesis can condense to form grains in circumstellar outflows that inject the grains into the interstellar medium (ISM). Once in the ISM, the grains may be further processed by supernova shocks and in molecular clouds before being incorporated into young stars and planetary systems during star formation in the clouds. Solids believed to be the remnants of the formation phase are found in our own solar system and around some other main‐sequence stars. Wolf‐Rayet stars, AGB stars, novae, and supernovae appear to be capable of expelling copious quantities of gas‐phase condensables. Observations of these objects show that at least some of this gas condenses into grains. The remaining gas from the outflows from these stars may be subsequently processed onto grains in the cores of dense molecular clouds.
Grains in circumstellar shells, the ISM, and the solar system are similar in general mineral content. The same four basic mineral types—amorphous carbon, silicates, silicon carbide, and hydrocarbons—are present in all three environments. There are, however, significant differences between the grains in these diverse environments (Gehrz 1989). Grains in the general ISM appear to be much smaller than their circumstellar counterparts, and grains in some comets were apparently annealed at high temperatures. Such annealing, for example, may explain the presence of spectral structure caused by emission from glassy silicate grains in comet Hale‐Bopp C/1995 01 (Hayward & Hanner 1997). Nonetheless, several properties of comet dust and meteorite inclusions suggest that grains made in circumstellar shells ("stardust") were incorporated directly into solid bodies in the primitive solar nebula. For example, some meteorites contain small grain inclusions with chemical abundance anomalies that strongly suggest that these grains ("presolar grains") condensed in the ejecta of supernovae, novae, WR stars, and red giant AGB stars. This would imply that some grains may have survived from the circumstellar to the solar environments relatively unchanged. On the other hand, it has been argued on theoretical grounds that it is difficult for stardust to survive destruction either by supernova shocks in the ISM (Seab 1987) or by high temperatures during the early evolution of young stellar objects (Boss 1988). Although they contribute only a small amount of stardust to the ISM of a galaxy, classical novae are the only objects in which it has been possible to observe directly all aspects of the temporal development of circumstellar grain formation events on a frequent basis. A number of IR studies of classical novae have been reported (Gehrz 1988, 1990, 1993, 1995). The IR temporal development of CO novae (explosions on carbon‐oxygen white dwarfs) is governed by the formation and evolution of the grains in the outflow, and IR observations have been used to characterize the physical conditions under which grains nucleate and grow as they are expelled into the ISM. Neon novae, also referred to here as ONeMg novae (explosions on oxygen‐neon‐magnesium white dwarfs with M*≳1.2 M⊙), produce little or no dust but expel large quantities of gas that can be observed through near‐ and mid‐IR forbidden‐line radiation from highly ionized "coronal" atomic states. Analysis of the chemical abundances of the gaseous ejecta of several recent neon novae strongly suggest that their ejecta may be associated with some of the chemical abundance anomalies seen in solar system meteorite grain inclusions. In this review, we concentrate specifically on the stardust and gas‐phase chemical anomalies that may have been contributed to the Galactic ISM by classical novae.
Novae are concentrated toward the center of the Galaxy, where they suffer obscuration by interstellar dust. Therefore, some of their global properties have only recently been revealed from studies of novae in nearby galaxies. Novae are generally associated with an older stellar population in galaxies, since both the spatial distribution and the relative frequency of novae are well correlated with the IR K‐band luminosity (Ciardullo et al. 1990) and the majority of novae appear to be associated with a disk population (Hatano el al. 1997). The influence of nova ejecta on the ISM is, therefore, generally global, except for the case of novae occurring on the most massive WDs. Della Valle et al. (1992) have recently studied the spatial distribution of a "fiducial" sample of Galactic classical novae. They find evidence that the more massive ONeMg novae are concentrated more tightly to the Galactic plane than are the lower mass carbon‐oxygen (CO) novae. This is consistent with theoretical expectations (Truran & Livio 1986; Ritter et al. 1991; Livio & Truran 1994; Starrfield et al. 1998). These findings would also suggest that ONeMg novae may be particularly important in contributing to chemical anomalies in regions of star formation near the Galactic plane. Roughly 30–40 novae per year occur in the Galaxy (Arp 1956; Ciardullo et al. 1987; Capaccioli et al. 1989; Shafter 1997), a significant fraction of which are known to eject material at high velocities during outburst, with this material usually enriched in elements heavier than He.
2. NATURE OF THE OUTBURST
Our understanding of the classical nova phenomenon has advanced rapidly over the past two decades because of significant progress in theoretical modeling of the outbursts and, in particular, the acquisition of an expanding volume of observational data describing novae in outburst. Observations of novae are now available in virtually all interesting wavelength ranges (Starrfield 1988, 1989, 1990, 1995). Recent observations have served both to constrain the theoretical models and to provide a quantitative measure of the influence of classical nova explosions on the surrounding ISM.
It is now commonly understood that a nova outburst results from a thermonuclear runaway (TNR) on the surface of a white dwarf (WD) accreting matter in a close binary system. The companion stars in these cataclysmic variable (CV) systems are observed to be late‐type stars that are filling their Roche lobes and passing matter through the inner Lagrangian point, via a disk, onto the surface of the WD. Accumulation of a hydrogen‐rich envelope on the WD continues until a critical pressure is achieved at the base of the accreted envelope and a TNR ensues. There follows: (1) a rapid rise of the luminosity to a maximum value that approaches (and, for all "fast" novae, exceeds) the Eddington limit; (2) a period over which the rekindled hydrogen‐burning shell powers the system at approximately constant bolometric luminosity; and (3) a gradual return of the system to its preoutburst state. The detailed features of such outbursts have been reviewed by a number of authors (Truran 1982; Gehrz 1988; Starrfield 1988, 1989, 1990, 1995; Shara 1989; Livio 1993).
2.1. Physical Quantities That Characterize the Outburst
Some relevant properties of the WDs in nova binary systems are presented in Table 1. All masses here are given in units of solar masses, M⊙. Note, particularly, that the tabulated values are listed as a function of the mass of the underlying WD; this simply reflects the fact that it is this mass dependence that is most critical to defining the observable properties of classical nova systems in and out of outburst. The WD radii shown here are obtained from the mass‐radius relation of P. Eggleton (1982, private communication), following the discussion of Truran & Livio (1986). A TNR in a nova envelope occurs when a critical pressure Pcrit, which we take to be independent of the WD mass, is achieved at the base of the accreted hydrogen envelope. This pressure is related to the radius and to the mass of accreted matter (the "envelope mass" Menvl) by the relation (Fujimoto 1982; MacDonald 1983; Prialnik et al. 1982):

where P is in dynes cm−2, G = 6.67 × 10-8 dynes cm2 g−2, MWD and Menvl are in grams, and RWD is in centimeters. The tabulated values of Menvl are derived from this expression for the specific choice of a critical pressure, Pcrit = 2 × 1019 dynes cm−2, consistent with numerical hydrodynamic models of nova explosions. The quantities εb and Eb are, respectively, the gravitational binding energy per gram (in ergs g−1) and the total gravitational binding energy (in ergs) of this accreted envelope.
![]() |
Hydrodynamic studies of nova eruptions predict that, following the TNR (and a brief, transient phase of evolution at super‐Eddington luminosity experienced by all fast novae; Truran 1982), shell hydrogen burning of the residual matter defines a phase of evolution characterized by a constant bolometric luminosity. The total luminosity of the burning shell during this phase is thought to be well represented by the core‐mass‐luminosity relation of Paczyński (1971; see also Iben & Tutukov 1989):

which approaches the Eddington luminosity

with increasing WD mass. In equations (2) and (3), LPacz and LEdd are in L⊙, MWD is in M⊙, the speed of light c = 2.9979 × 1010 cm s−1, and κT, the Thomson opacity for scattering by free electrons, is 0.3975 cm2 g−1 for hydrogen. LPacz and LEdd are both included in Table 1. From a knowledge of the nuclear shell‐burning luminosity LPacz and the fact that for matter of solar hydrogen concentration the total energy available per gram from nuclear burning is ∼4.7 × 1018 ergs g−1, we can readily compute two additional quantities of interest: the effective rate of mass consumption via nuclear burning dMnucl/dt =M˙nucl in units of M⊙ yr−1 and the "nuclear timescale" τnucl, where

In equation (4), τnucl is in years and the quantities Menvl and LPacz are in solar units. The need for some additional mechanism of mass loss is evident from these data (see Table 1). Calculations show that while the turnoff of a nova demands the virtual exhaustion of the available nuclear fuel, the nuclear timescales for typical TNRs on WDs with masses of ∼1–1.2 M⊙ are much longer than the observed timescales for the return of classical novae to minimum following their outbursts (Orio 1998). In our subsequent discussion, we will examine the possibility that the composition of the ejected matter may vary considerably from the early to the late stages of mass ejection. Following the return of a nova to minimum, it is assumed that the process of mass transfer will recommence and the progressive buildup of a hydrogen layer on the WD will continue until sufficient mass is assembled to trigger the next TNR. The time required to accumulate the required envelope mass Menvl, the "recurrence timescale" τrecur, assuming a constant accretion rate M˙acc ≈ 10−9 M⊙ yr−1 (see King 1989) to be characteristic of all systems, is also presented in Table 1.
A factor of particular relevance to the interpretation of the abundance patterns that are found to characterize nova ejecta is the relative frequency of occurrence of novae as a function of the mass of the underlying WD. The relative frequencies of occurrence calculated by Truran & Livio (1986; see also Politano et al. 1990; Ritter et al. 1991) are presented in Table 1 as the quantity f. In particular, we call attention to the high frequencies of occurrence of systems involving high‐mass WDs (M*≳1.2 M⊙). This is a consequence of a strong selection effect. The increased gravitational potential of high‐mass WDs—as evidenced in the higher values of the binding energy per gram included in Table 1—is such that the critical pressure needed to trigger the TNR is achieved at a significantly lower accreted envelope mass Menvl. In fact, it follows from the mass‐radius relationship for WDs (M1/3WD RWD = constant) that Menvl ∝ Pcrit M-7/3WD. Therefore, massive WDs require significantly less accreted matter to trigger a TNR and experience recurring outbursts on much shorter timescales. Thus, while low‐mass WDs are far more abundant in the Galaxy, the masses of the WDs in those nova systems observed in outburst tend to be significantly larger. Truran & Livio (1986; see also Livio & Truran 1994) estimated the mean mass of the WDs in systems observed in outburst to be ∼1.1–1.2 M⊙, as opposed to the mean masses ∼0.6 M⊙ observed for single WDs.
The importance of this selection effect is emphasized when considering the relative frequencies of CO and ONeMg WDs. Theoretical expectations are that CO WDs range in mass up to ≈1.2 M⊙, while WDs of masses ≳1.2–1.3 M⊙ are expected for ONeMg WDs (Iben & Tutukov 1985). This selection effect thus provides a natural understanding of the high frequency of ONeMg WDs identified in nova systems. This will become apparent in our subsequent discussions of the observed abundances of the ejecta of recent novae (see Tables 2 and 6).
![]() |
Theoretical models and general energy considerations indicate that most of the accreted matter is ultimately ejected in the outburst and thus returned to the ISM. The mean mass returned by a nova outburst to the ISM is of the order of 2 × 10-4 M⊙ (for the occurrence frequencies calculated by Truran & Livio 1986). In fact, from our Table 1 we can readily see that this mass estimate is dictated rather by the greater ejected masses characteristic of systems involving lower mass WDs. We will return to this question when we consider possible sources and levels of production of various types of abundance and grain anomalies. Using the observed nova rate of 35 ± 11 yr−1 in our Galaxy (Shafter 1997), it follows that novae introduce approximately 7 × 10-3 M⊙ yr−1 of processed matter into the ISM. Supernovae, occurring at a rate of one every 50 yr and ejecting approximately 3 M⊙ per event, introduce roughly 0.06 M⊙ yr−1 of processed matter into the ISM. Therefore, if novae are to contribute significantly to nucleosynthesis, the levels of overproduction of heavy elements in novae must be of order 10 times the levels characteristic of supernova ejecta. The argument above may have to be revised in favor of novae if nova ejecta are far more massive than heretofore believed (Saizar & Ferland 1994; Ferland 1998). We emphasize this crucial question of the global contribution of novae and will revisit it several times in the discussion that follows.
The mass estimate provided here also allows us to determine the energy contributions of individual nova events to the ISM. Velocity determinations for the principal ejecta of novae lie in the range 400–2000 km s−1, although some (seemingly small) fraction of the material appears to be ejected at velocities as high as 10,000 km s−1. This implies that the total output kinetic energy from novae will fall in the range 1045–1047 ergs yr−1, well below values typical of supernovae, and that novae do not constitute a significant source of energy for the heating of the ISM.
Finally, spectroscopic studies of classical novae have revealed two extremely important features: (1) the presence of extremely large concentrations of C, N, and O nuclei and, more recently, of O, Ne, Mg, and Al nuclei in the ejecta; and (2) the formation of grains in a substantial number of the slower novae. The issue of grain formation and its implications will be addressed in a subsequent section. It is appropriate at this stage of the discussion, however, to examine briefly the theoretical implications of the heavy‐element enrichments. We will be concerned both with the total levels of overproduction of heavy elements in novae and with the relative contributions from the two broad classes of CO and ONeMg novae, respectively.
2.2. Determination of Physical Quantities from IR Observations
IR observations are particularly useful for providing definitive measurements of many of the physical parameters that describe nova TNRs and the resulting ejecta (Bode & Evans 1989; Gehrz 1988, 1990, 1993, 1995). Table 3 of this paper (after Gehrz 1995) summarizes the way in which these parameters can be derived from the IR measurements. The quantities involved in the initial determinations of the abundances of nova shells using optical/UV lines are shown in Table 4. Values for some of these parameters from IR studies of 25 recent novae, for which sufficiently extensive observations have been obtained, are given in Tables 5 and 6. Two fundamentally different types of nova events, which we refer to hereafter as CO and ONeMg novae, can be distinguished by IR measurements (see Gehrz 1995; Gehrz et al. 1995b). Both CO and neon novae behave similarly in the IR immediately following the outburst. Hot gas expelled in the explosion is initially seen as an expanding photosphere or "fireball," but free‐free and line emission dominate the IR as soon as the expanding material becomes optically thin. At this time, the development of CO and ONeMg novae diverges. The free‐free phase in a CO nova is typically followed by dust formation, characterized by a sudden extinction of the visible light accompanied by rising IR emission, about 30–80 days after outburst. In ONeMg novae, the free‐free phase evolves into a coronal emission‐line phase.
![]() |
![]() |
![]() |
![]() |
Many CO novae condense enough carbon dust to produce a visually optically thick carbon dust "cocoon" that acts as a calorimeter by completely obscuring the central engine at short wavelengths while reradiating the entire luminosity of the central engine in the thermal IR. These cases, typified by NQ Vul (Ney & Hatfield 1978) and V705 Cas (Gehrz et al. 1995a; Mason et al. 1997), are particularly valuable for assessing the duration of the constant luminosity phase of the central engine referred to above. The ejected shells of the CO novae tend to be of high mass and to have expansion velocities at the low end of the range observed in novae, although CO novae with heavy‐element–enriched envelopes (e.g., V1668 Cyg/1978) can be "fast" novae as well. TNRs on more massive ONeMg WDs produce lower mass, high‐velocity shells in which the shell density may be too low at the base of the condensation zone to enable the production of appreciable amounts of dust. Note that it is very likely the mass of the WD that is the critical factor: CO novae are expected to involve WDs of mass ≲1.2 M⊙, while ONeMg novae probably involve WDs of mass ≳1.2 M⊙ (see Table 1). The fact that CO novae are the more prolific producers of dust is most likely a consequence of the fact that these novae, typically involving lower mass WDs than do neon novae, eject substantially more mass at lower velocities.
IR observations of the dust and gas in novae are becoming an important source of abundance information. Recent studies have particularly emphasized evaluations of the abundances of elements such as C, N, O, Ne, Na, Mg, Al, Si, and S in the ejecta to determine the degree to which classical novae participate in the chemical evolution of the Galaxy (see Gehrz, Truran, & Williams 1993, hereafter Paper I). CO novae produce silicates, silicon carbide, carbon, and hydrocarbons. Several novae have condensed all four types of grains in a single eruption, suggesting that novae may have a complex temperature, density, and abundance structure in their ejecta. Theoretical models (Timmes & Truran 1997) indeed indicate that there can exist quite different and distinctive elemental and isotopic composition gradients across the ejected envelopes of novae. Infrared observations of the dust can be used to obtain measurements of the abundances of C and other grain constituents such as Mg, Si, O, and Fe.
ONeMg novae are distinguished in the IR by the formation, soon after outburst, of strong IR forbidden emission lines that include a number in the near‐IR as well as those from [Ne vi] at 7.62 μm and [Ne ii] at 12.8 μm. The exceptionally powerful [Ne ii] emission from the prototype coronal nova QU Vul/1984 #2 led Gehrz, Grasdalen, & Hackwell (1985) to term these "neon" novae. Abundance estimates for Al, Ar, Ca, Na, Ne, S, and Si in the ejecta can be determined from measurements of forbidden‐line emission strengths in the 2–40 μm spectral region. We summarize later the values (see Tables 5 and 6) that have been measured using IR techniques for physical parameters of the nova outburst (see Table 3) and abundances of nova ejecta. Recent results on the bright novae V1974 Cyg/1992 (a neon nova) and V705 Cas/1993 (an extreme DQ Her–type CO nova) are used to illustrate the techniques.
3. THERMONUCLEAR PRODUCTION OF HEAVY NUCLIDES
Nucleosynthesis associated with nova events is limited to the effects of the proton‐induced reactions that can proceed at the temperatures of 1.8 × 108 to 3 × 108 K typically achieved in their hydrogen‐burning shells. Higher temperatures are not generally attainable because of the lifting of degeneracy in the outer layers of the WD. CNO cycle hydrogen burning will, of course, act to redistribute these nuclei and thereby alter the concentrations of the isotopes of carbon, nitrogen, and oxygen. Similarly, the NeNa and MgAl cycles will act to alter the isotopic compositions of neon and magnesium. At the highest temperatures achieved on ONeMg WDs, nuclear transformations can also lead to the production of enhanced concentrations of S and Ar. The observational situation regarding the abundances of these and heavier nuclei in nova ejecta, and the implications of the observed enrichments of heavy elements will be addressed in the following sections of this paper. In this section, we review the possible role of novae in the synthesis of7Li and the rarer isotopes of the CNO elements, as well as in the formation of significant abundances of the radioactive nuclei22Na and 26Al.
It is important to recognize in advance that the relative contribution of novae to Galactic nucleosynthesis is small and that detailed predictions regarding their contributions are quite uncertain. We do not know with confidence either the rate of nova outbursts as a function of time over the history of our Galaxy or the average mass ejected per outburst. Nonetheless, the total amount of processed matter contributed by novae to the ISM may be roughly estimated by assuming: (1) the current rate of nova events is 35 ± 11 yr−1 (Arp 1956; Capaccioli et al. 1989; Shafter 1997); (2) the rate has remained constant over the lifetime ∼1010 yr of the Galactic disk; and (3) the average mass ejected per nova event is ∼10−4 M⊙ (see the discussion in §2). This implies a total mass ∼4 × 107 M⊙. By comparison, supernovae occurring at a rate of one per 50 yr and ejecting ∼2 M⊙ per event would have processed 4 × 108 M⊙ during the same period. Since the mass processed through novae represents only a fraction (≈1/250) of the mass of interstellar matter (∼1010 M⊙) in our Galaxy, it follows that significant contributions require enrichments, relative to solar system abundances, in nova ejecta of factors of more than 250. This would apparently rule out the possibility that novae are important contributors to Galactic abundances of the dominant isotopes of carbon and oxygen, and probably of nitrogen as well. Novae may, however, produce important amounts of the rarer CNO isotopes 13C and, particularly,15N and 17O. In fact, Timmes & Truran (1997) find that novae may well be the major Galactic source of 15N and 17O.
Classical nova outbursts provide an environment in which hydrogen‐burning reactions proceed on carbon, nitrogen, oxygen, and heavier nuclei, at high temperatures and densities on a dynamic timescale. For such conditions, the production of potentially interesting and detectable abundance levels of the radioactive isotopes 22Na and 26Al can also occur, and novae are believed by many to be potentially important nucleosynthesis sites for these elements. Weiss & Truran (1990; see also Nofar, Shaviv, & Starrfield 1991) have modeled the nucleosynthesis accompanying nova explosions for representative temperature histories extracted from hydrodynamic models, utilizing a large nuclear reaction network. Their results confirm the earlier findings of Hillebrandt & Thielemann (1982) and Wiescher et al. (1986; see also Van Wormer et al. 1994) that extremely low levels of both 22Na and 26Al are formed in nova envelopes of initial heavy‐element composition comparable to that of solar system matter. This implies both that we should not expect to find detectable levels of 22Na in the ejecta of novae with relatively low heavy‐element concentrations and that such novae do not contribute significantly to the abundance of 26Al in the Galaxy. Moreover, enriched CNO concentrations alone do not guarantee any significantly increased 22Na and26Al production. The calculations by Weiss & Truran (1990) predict low concentrations of 22Na and 26Al, even with the assumption of matter enriched to ZCNO = 0.25. As anticipated, greatly increased 22Na and26Al production can result for envelopes characterized by substantial initial enrichments of elements in the range from neon to aluminum. Since these abundance enrichments are presumed to arise as a consequence of the dredge‐up of core matter (see, e.g., Starrfield 1989; Livio & Truran 1990; Starrfield et al. 1998), it follows that such systems involve more massive ONeMg WDs.
Theoretical estimates (Truran & Livio 1986; Truran 1990; Livio & Truran 1994) and spectroscopic analyses of the ejecta of recent novae (Table 2) both indicate that such neon‐rich novae comprise approximately 25%–40% of all observed classical nova outbursts. For the explosive hydrogen‐burning conditions that characterize novae, burning by means of the NeNa and MgAl hydrogen‐burning sequences can yield substantial concentrations of22Na and 26Al. Specifically, for the choice of an initial composition consisting of matter enriched to a level Z = 0.25 in the products of stellar carbon burning (Arnett & Truran 1969), the calculations of Weiss & Truran (1990) predict that the abundance levels of 22Na and 26Al can be 1–2 orders of magnitude larger than for the case of matter of initial solar composition. They conclude that such neon‐rich novae may represent an important source of 26Al in our Galaxy. Future observations of the distribution of 26Al in the Galaxy may provide constraints upon the nature of the progenitors and, perhaps, indicate whether red giants, novae, or supernovae represent the dominant source.
The general trends identified in these systematic nucleosynthesis studies have since been confirmed by calculations of nucleosynthesis coupled directly to hydrodynamic models. The extensive models by Prialnik & Kovetz (1995), for TNRs occurring on CO WDs, yield the expected range of variations of the CNO isotopes in their ejecta. Politano et al. (1995) calculated the nucleosynthesis accompanying nova outbursts on ONeMg WDs of masses 1.0, 1.25, and 1.35 M⊙. Modifications of the CNO isotopic patterns, enrichments of sulfur, and significant abundances of22Na and 26Al were found to be associated with such outbursts. New evolutionary sequences for novae involving 1.25 M⊙ ONeMg WDs, using updated reaction rates and opacities (Starrfield et al. 1998) reveal similar overall trends, but somewhat reduced levels of26Al production. Building upon the extensive survey of nucleosynthesis involving ONeMg WDs by Wanajoh (1996), Wanajoh, Nomoto, & Truran (1997) have demonstrated that detailed abundance patterns can provide clues to the masses of the underlying WDs in ONeMg systems which, themselves, can be quite unambiguously identified by their low values for the element abundance ratio (C + N + O)/(Ne + heavier elements), as shown in Table 2. Finally, two‐dimensional hydrodynamic models of the stages of nova TNRs through maximum energy generation (Glasner, Livne, & Truran 1996, 1997) suggest that convective overshooting could contribute to the enrichment of nova envelopes in CO or ONeMg core matter.
4. NOVA PRODUCTION OF INTERESTING RADIOACTIVITIES
Theoretical models indicate that novae may be expected to form interesting amounts of 7Be (τ1/2 = 53 days) and 22Na (τ1/2 = 2.7 yr), the lifetimes of which are comparable to those of novae in outburst, as well as the longer lived 26Al (τ1/2 = 7.2 × 105 yr). The decays of 7Be and 22Na can yield potentially detectable levels of γ‐ray emission for relatively nearby objects. The detection of these γ‐rays would provide an important confirmation of essential features of the TNR model. Novae can also produce a significant fraction of the 26Al that is observed to exist in Galactic matter. The 22Ne (Black 1972) and 26Mg (Truran 1985) anomalies found in meteorites are especially interesting in the context of classical novae in view of theoretical predictions that large amounts of 22Na and26Al can be produced in outbursts involving ONeMg WDs (Weiss & Truran 1990; Nofar et al. 1991). Current predictions regarding 22Na, 26Al, and 7Be production in novae are reviewed in this section.
4.1. The22 Ne (neon‐E) Anomaly:22 Na Production and Decay
The radioisotope 22Na decays to22Ne via the β‐decay reaction 22Na → 22Ne + e+ + ν, leading to the emission of 1.275 MeV γ‐rays. The mean life of the reaction is only 3.75 yr, so that novae will be short‐lived sources of γ‐rays from 22Na decay, and the Galactic background should be very anisotropic, with strong peaks in the direction of the most recent novae. No detections of 1.275 MeV γ‐rays have been made to this date, but several upper limits were placed on the diffuse Galactic emission and specific nova events. Higdon & Fowler (1987) concluded that limits to the diffuse Galactic 1.275 MeV flux suggested a production of less than 6 × 10−7 M⊙ of 22Na per nova event. Leventhal, MacCallum, & Watts (1977), using observations from a balloon‐borne γ‐ray detector, reported that V1500 Cyg/1975 must have produced less than 10−6 M⊙ of22Na.
Despite the fact that no detections have yet been made, the concentrations of 22Na predicted for the ejecta of novae involving ONeMg WDs are sufficiently high that we may expect relatively nearby novae to produce flux levels of 22Na decay γ‐rays that are detectable at Earth. The calculations of Weiss & Truran (1990) estimate that the average yield of 22Na per ONeMg outburst should be between 10−8 and 10−7 M⊙, a level that has been confirmed as well by calculations by Politano et al. (1995) and Starrfield et al. (1998). The flux of22Na 1.275 MeV γ‐rays from an individual nova event is

where Mej is the total mass ejected in the nova event, X22 is the mass fraction of22Na, and D is the distance to the nova in kiloparsecs. A level of production of 22Na of X22≳10-3 is consistent with the calculations of Starrfield et al. (1998). Such novae at distances of ≤1 kpc should thus be considered as promising targets for Compton Gamma Ray Observatory (CGRO). By comparison, we note that the quoted CGRO upper limit on 1.275 MeV line emission from V1974 Cyg/1992 of ∼10−4 cm−2 s−1, assuming a distance of 2.5 kpc, corresponds to an ejected 22Na mass of ∼8 × 10−8 M⊙ (Shrader et al. 1994). It is reasonable to assume that the large distance to V1974 Cyg/1992 was the critical factor in explaining the lack of a detection. The problem may be more serious for V838 Her 1991, since the 3 σ upper limit to the 1.275 MeV flux measured with CGRO (Iyudin et al. 1995) is a factor of ≈2.7 lower than the level predicted by Starrfield et al. (1992) based on the ejected mass of ≈7 × 10−5 (Woodward et al. 1992). A related issue concerns the theoretically predicted masses of the accreted (and ejected) envelopes of these "neon" novae as compared to the values that have been observed (Starrfield et al. 1998).
We emphasize the importance of the detection of 22Na γ‐rays from novae as a means of imposing important constraints on theoretical models of nova outbursts and their role in Galactic nucleosynthesis. In this context, however, it is important to recognize that the 22Na concentrations produced in novae are quite generally insufficient to account for the observed abundance of its decay product 22Ne in the Galaxy. Truran & Hillebrandt (1986) showed that the observational upper limit on the γ‐ray flux (Mahoney et al. 1982, 1984) is such that the total amount of 22Na ever formed constitutes less than approximately 1% of the mass of 22Ne in the Galaxy today. Thus, most of the mass of 22Ne in the Galaxy is actually incorporated into the ISM directly as 22Ne. On the other hand, novae and other astrophysical events may make interesting amounts of 22Na with respect, for example, to the generation of the Ne‐E anomaly in meteorites. Presuming this anomaly to be caused by β‐decay of 22Na, the short mean life for this decay implies that the parent 22Na of the22Ne present in grains must have been trapped in the grains very shortly after its formation at the nucleosynthesis site.
4.2. 26 Mg Anomaly:26 Al Production and Decay
The principal question of interest regarding 26Al is whether novae can have contributed significantly to the production of the ∼2 M⊙ of 26Al in the Galaxy that is required to explain the 1.809 MeV γ‐ray line emissions from the decay of 26Al to 26Mg first reported by Mahoney et al. (1982, 1984) with the High Energy Astronomical Observatory (HEAO) and by Diehl et al. (1995a, 1995b) with CGRO. Following the arguments presented by Weiss & Truran (1990) and Nofar et al. (1991), the mass of26Al in the Galaxy that is attributable to nova explosions can be estimated from the relationship

Here we have assumed a rate of occurrence of nova events of 35 yr−1, a fraction 0.50 of all nova events that produce26Al f26, a 26Al mass fraction X26 in the ejecta of 10−3, and an ejected mass of 10−4 M⊙ per event for the ONeMg systems. We have chosen the rather large value for the ejected mass of 10−4 per event based upon the improved accuracy of recent observations of the best‐studied novae including those of QU Vul/1984 #2 and V1974 Cyg/1992. The result obtained using equation (6) is in agreement with the approximately 2 M⊙ required to account for the γ‐ray observations (see Diehl et al. 1995a, 1995b) and is comparable with the range of 1.78–2.14 M⊙ Myr−1 for supernovae quoted by Timmes, Woosley, & Weaver (1993). Shara (1994) and Prialnik & Shara (1995) have speculated that even low‐mass CO WDs may produce significant quantities of26Al, although this scenario does not agree with observations. If this is the case, then classical novae may indeed be competitive with supernovae as a source of 26Al on a Galactic scale. Note, however, that the recent studies by José, Hernanz, & Coc (1997) and by Starrfield et al. (1998) find relatively low levels of production of26Al for both CO and ONeMg WDs.
As in the case of22Na, classical novae also appear to be a promising source of26Al, at least on local scales where an isolated collapsing cloud could be affected by ejecta from a nearby nova. This may have been the case for the solar system (Clayton 1982; Prantzos 1993). There is chemical evidence that the 26Al that produced the 26Mg anomaly in meteorites was alive in the primitive solar system, implying that the parent nova (if a nova and not a red giant or a supernova was, indeed, the source) was relatively nearby (see the discussion by Cameron 1984). The decay of 26Al to 26Mg by the reaction26Al → 26Mg + e+ + ν has a mean life of 106 yr. This long mean life would enable ejecta from many eruptions of a single nova system to inject live 26Al into the primitive solar system during its formation. The long mean life also implies that the Galactic background at 1.809 MeV from novae should be fairly smooth, with components from the disk population as is observed. Further information concerning the distribution of 26Al in the Galaxy may ultimately be able to distinguish between supernovae, novae, and red giant stars as sources of 26Al.
4.3. 7 Be Production and Decay
A question of considerable interest to nucleosynthesis and cosmology is whether nova explosions are an important site for7Li production. The production of 7Li can indeed occur by electron capture onto 7Be in the TNRs that cause the outbursts of classical novae (Arnould & Nørgaard 1975; Starrfield et al. 1978; Hernanz et al. 1996). The mode of 7Li production here is similar to that in red giants:7Be is carried outward by convection to cooler regions of the envelope on a sufficiently rapid timescale to ensure that it will not be destroyed via 7Be (p, e+γ) 8B before β capture can occur to expedite the 7Be (e−, ν)7Li reaction. The total mass of 7Li ejected is a sensitive function of the conditions achieved in the outburst and may therefore be expected to vary from event to event. Model calculations (Starrfield et al. 1978; Hernanz et al. 1996) predict that7Li enrichments of factors of up to ∼102 to 103 may characterize the ejecta of novae under some circumstances. The critical dependencies with regard to 7Li production are on the temperature history of the matter and the convective character of the envelope over the course of the TNR (both of which may be a function of the speed class of the nova). The initial 3He concentration of the envelope matter is also of interest, since much of the3He initially present in the envelope may be converted readily to 7Be. For the specific (and favorable) choice of a factor 1000 enrichment, we obtain the following expression for the expected flux of γ‐rays from 7Be decay:
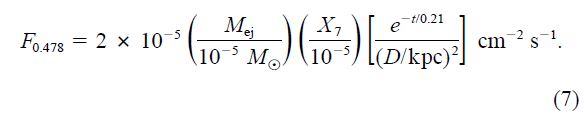
The integrated contributions of classical novae to the abundance of7Li in Galactic matter cannot be estimated very reliably because of uncertainties in our knowledge of the properties of the progenitors of classical nova systems and of their rate of formation over the past history of the Galaxy. Nevertheless, a statement regarding production of 7Li in novae is possible. The theoretical models proposed by Politano et al. (1995) and Starrfield et al. (1998) predict average enrichment factors for the rare isotopes 15N and17O that are approximately 10 times that of 7Li. If these relative production ratios are representative of classical nova systems, and novae are indeed the major source of 15N and17O in the Galaxy (Timmes & Truran 1997), then it must follow that novae can account for, at best, only ∼10–20% of the 7Li in the Galaxy if we assume that 15N and 17O have their origin entirely in nova events. Further detailed calculations of nucleosynthesis coupled to hydrodynamic models of novae are required to clarify the situation for7Li. In view of the importance of questions concerning7Li production and destruction mechanisms, with regard to the implied constraints on the cosmological production of 7Li, a new examination of the problem of 7Li synthesis in novae seems appropriate. In particular, it may prove interesting to explore the effects of higher initial 3He.
5. ULTRAVIOLET, OPTICAL, AND INFRARED SPECTROSCOPIC MEASUREMENT OF THE ABUNDANCES OF HEAVY NUCLIDES
The study of novae ejecta is central to the understanding of the nature of the outburst. Gaseous shells having a range in mass from Mej ∼ 10−7 to 10−3 M⊙ are ejected with velocities of 400–10,000 km s−1 from each outburst, and this material may be an important nucleosynthesis source for certain elements and/or isotopes in the ISM. The fact that some novae form considerable quantities of dust soon after outburst also may have consequences for star formation in the Galactic disk. The relative fraction of the ejected mass that precipitates out of the gas into grains is difficult to ascertain, but, judging from the sudden decrease in the line emission from certain elements at the time that dust condensation occurs, it is substantial for some novae. Significant progress in our understanding of the nova phenomenon has taken place over the past decade as a consequence of observational studies at UV, optical, and IR wavelengths. We review below recent determinations of abundances in novae ejecta.
5.1. Gas‐Phase Abundances: The Optically Thick Phase
In recent years, owing largely to the rapid development of UV, optical, and IR technology, numerous high‐quality spectra have been obtained for novae in outburst across a broad wavelength range. Especially important in this growing archive have been data obtained during the early stages of the outburst. The early expansion stage is a time when the ejected envelope is extended but still mostly optically thick and, therefore, an accurate treatment of spectrum formation requires the use of a spherical, expanding, model atmosphere code. Such studies were begun only in the past 5 years. The importance of analyzing optically thick nova spectra is that they contain a wealth of information about the temperature and density distribution in the ejected material. They also sample a large range of velocities and depths, as compared with the optically thin stage, and can be used to determine the elemental abundances, the expansion velocities, and the energy budget in the outer layers. Reliable determinations of all the parameters available from spectroscopic analyses are now providing significant insight into the physics of the explosion and considerably constraining the hydrodynamic simulations.
The current studies of optically thick novae have been facilitated by the development of a computer code that calculates line‐blanketed, expanding, spherical, non‐LTE model atmospheres (Hauschildt et al. 1992, 1994a, 1994b, 1995, 1996, 1997). The code solves the special relativistic radiative transfer equation for over 100,000 frequencies in the comoving frame and does not use the Sobolev approximation, which is not valid for atmospheres with large numbers of overlapping lines (Baron, Hauschildt, & Mezzacappa 1995). These atmospheres treat the millions of lines of the iron peak elements (neutral, singly, and doubly ionized) in full non‐LTE. This is required in order to achieve realistic results (Hauschildt et al. 1997; Schwarz et al. 1997a).
It should be emphasized that abundances determined with this method are completely independent from those obtained with the method normally used to determine elemental abundances for novae, that is, the analyses of the nebular emission lines at late stages in the outburst (see the next subsection). Comparisons of the abundances obtained from these two methods, for the same nova, provide an important and unique check on each method.
Studies of optically thick spectra are important for a number of reasons. Since the ejecta are expanding according to a simple linear velocity law, this means that the outermost material, which is moving fastest, becomes transparent first. Therefore, in the most opaque stages of the outburst and throughout the period when the absorption spectrum is weakening, the photosphere recedes in the ejecta through layers that may not be visible in the later, optically thin spectra. The structure of the atmosphere is, however, very different from that of a static plane‐parallel atmosphere. First, there is no real photosphere; hence, there is no effective temperature for the model. The continuum is more correctly characterized by a color temperature. Second, the steep density gradients resulting from the expansion and the large curvature of the atmosphere combine to complicate the radiative transfer. Third, there is no a priori reason for thinking that the abundances of all elements, especially those synthesized during the explosion, should be uniformly distributed in the ejecta. With spectral modeling of the early stages, such assumptions can be examined.
A nova model atmosphere is characterized by the following parameters (plus others): (1) the radius where either the optical depth in absorption or extinction at 5000 Å is unity; (2) the model temperature, Tmodel, which is defined via the luminosity and the radius (note that the effective temperature is not defined for a spherical, expanding atmosphere); (3) the density law (typically ρ∝r-N, but it can be chosen arbitrarily, e.g., from a hydrodynamic calculation); (4) the rate of mass loss or the velocity field; (5) the density at the outer boundary; and (6) the elemental abundances. Items 3 and 4 will soon be replaced by a new study in which a stellar wind is included self‐consistently with the atmosphere.
The Hauschildt code was first used for a study of the spectral evolution of the slow CO nova PW Vul/1984 #1 (Hauschildt et al. 1992). This study used a radial density gradient with N = 3, derived from hydrodynamic calculations (Starrfield 1989, and references therein). This value of N is very different from the density exponent that characterizes the atmospheres of Type II supernovae (N ≈ 5–25; Baron et al. 1993, 1994, 1995). Such a small value for the density exponent in nova atmospheres has a number of important consequences. First, nova photospheres are more geometrically extended than those of virtually all other classes of stars so that the curvature terms in the radiative transfer equation are important in both line and continuum formation. Second, the low densities and the very nongray radiation field of the atmosphere cause large departures from LTE. Third, the electron temperatures span a huge range in nova atmospheres. For example, in an atmosphere with Tmodel = 15,000 K the electron temperatures range from 5000 to more than 30,000 K. This last result implies that a large number of ionization states should be present in the emergent spectrum, something that is actually observed and otherwise difficult to explain with conventional model atmospheres. If the temperatures are low enough, then molecules and dust can form in the outer layers even at optical maximum, which has also been observed (Sneden & Lambert 1975; Shore et al. 1994b). Finally, Hauschildt et al. (1992) also emphasized that the UV spectral features were not emission lines but, instead, were regions of transparency between overlapping absorption lines from the iron group elements (the "iron curtain": Starrfield & Shore 1994, 1995).
The next nova to be studied by this method was V1974 Cygni/1992. Because it was so bright at maximum (V ≈ 4) and was first discovered before visual maximum was reached, it was possible to obtain a very rapid time sequence of observations with IUE. Spectral syntheses were performed by Hauschildt et al. (1994a) for the first few UV and optical spectra obtained within about 18 hr of the report of the discovery of the nova. They found that the earliest spectrum could not be reproduced by the same shallow N ≈ 3 density profile as was used in the analysis of PW Vul. This spectrum could be reproduced, however, using a relatively steep,N ≈ 15, homologously expanding, shell with Tmodel = 11,000 K. This result implied that V1974 Cyg was in the "fireball" stage when first observed (see, e.g., Gehrz 1988). Hauschildt et al. also reported that the fits of the synthetic spectra to the observed spectra were improved if they used nonsolar abundances. The best agreement occurred when the metal abundances were increased by a factor of ≈2 and the CNO abundances were enhanced by a factor of ≈10. This was completely independent of the nebular abundances (Austin et al. 1996; Hayward et al. 1996) and is supported by the later results.
Combined IUE and optical spectra obtained after the initial recovery from UV minimum (Shore et al. 1993, 1994a, 1994b) were well reproduced with an atmosphere having N = 3 and Tmodel = 14,000 K. Hauschildt et al. (1994a) again found that it was necessary to increase the metal abundances by a factor of 2 and the CNO abundances by about a factor of 10 over solar values. The enhanced metal abundances were strong evidence for significant hydrogen depletion in the ejected shell as expected in a nova explosion since metal‐rich white dwarf core material is mixed into the shell during the TNR and hydrogen fusion reactions power the outburst. Since enhanced metal abundances were required to also fit the "fireball" spectrum, this implies that the envelope was very thoroughly mixed during the earliest stages of the outburst. These results demonstrate the power of spectral synthesis techniques.
The slow CO nova V705 Cas/1993 (Hauschildt et al. 1994a) formed dust during the optical decline just as the UV was approaching maximum brightness. This is only the second time that observations were obtained in the UV just as a nova was forming dust. The extensive coverage of spectra obtained with the IUE satellite made it possible to follow the spectral evolution of this nova through this phase. Hauschildt et al. (1994a) analyzed these spectra and found that Tmodel increased from discovery through the time that the dust began forming. Their study also demonstrated that radiation pressure was sufficient to eject the material even in a slow nova such as V705 Cas. This result has a direct impact on hydrodynamic modeling of the nova outburst. They were able to determine mass‐loss rates as a function of time, but this study will be improved when the spectra are reanalyzed with the radiatively driven wind plus atmosphere code now being developed (Aufdenberg, Hauschildt, & Shore 1998).
Although the fits to the observations were quite good and important results have been obtained, Hauschildt et al. have continued to improve and extend their code. The most important addition has been the treatment of numerous additional species in non‐LTE. In order to do this, it was necessary to develop new techniques to treat large numbers of species in non‐LTE self‐consistently with the radiation field (Hauschildt et al. 1994b, and references therein). Using newly derived operator‐splitting techniques (Hauschildt 1992, 1993), they first added Fe ii in non‐LTE (Hauschildt et al. 1996) and then a very large number of new elements and ionization states, which included the extremely important (for analyzing the observations) CNO lines (Hauschildt et al. 1997; Schwarz et al. 1997a, 1997b). These additions have markedly improved the correspondence between theory and observations (Schwarz et al. 1997a, 1997b). In other studies, Pistinner et al. (1995) showed both that fits of atmospheres to observed spectra were insensitive to the luminosity of the nova and, in addition, that the UV spectral range contained the most important information on the structure of the atmosphere. It is, therefore, unfortunate that NASA ended observations with the IUE satellite, since no other satellite (including HST) is capable of observing the UV during the critical first few days of the outburst.
Using the enhanced version of the Hauschildt code, Schwarz et al. (1997b) examined the early UV spectra of the CO nova OS And/1986 and found that the inclusion of Fe ii in non‐LTE significantly improved the fits of the atmospheres to observed spectra. They identified two subphases in the UV evolution of this nova. The earliest spectra, obtained only a few days after optical maximum, again show the typical pseudo‐continuum created by large numbers of overlapping absorption lines from the iron group elements. These data showed that the ejected material had solar metallicity, although there was marginal evidence that Mg was depleted. They also found that spectra obtained later in the evolution, when the temperatures had increased and the densities had dropped, showed a similar pseudo‐continuum. Superimposed on this continuum, however, were allowed, semiforbidden, and forbidden lines from a variety of species. They called this the "prenebular" phase and it reflects the increased importance of the optically thin outer layers in the spectral formation. Analyses of the spectra obtained in this phase again showed a solar composition.
Finally, Schwarz et al. (1997a) have analyzed the UV and optical spectra of LMC 1988 #1, which was a moderately fast, dust‐forming, CO nova. It was the first extragalactic nova to be observed with the IUE satellite. They found that their fits to the observed spectra were much improved by including Fe i to Fe iii lines in non‐LTE. The results of their analysis is that the CNO elements were about 10 times solar (Z = 1/3), and that the bolometric luminosity of this nova was approximately constant at ∼3 × 1038 ergs s−1 for the first 2 months of the outburst.
5.2. Gas‐Phase Abundances: The Optically Thin or Nebular Phase
As the nova ejecta expand, their density drops, continuum emission becomes less important, and forbidden lines from highly ionized species become progressively more pronounced in the spectrum. Although for many years it has been thought that this phase could be treated with standard techniques, devised for H ii regions or planetary nebulae, it now seems clear that more sophistication is needed. There are a number of reasons for this. First, the densities in the ejecta are still high, more than 107 cm−3, which is above the critical densities for collisional damping for many important ions (Osterbrock 1989). Second, there is a hot, underlying, photoionizing source whose radiation field is modified by transport through optically thin layers that governs the ionization and excitation of the important elements. Third, the expanding material is not spatially resolved so that a given spectrum effectively integrates over regions with widely different electron densities and temperatures. Fourth, the physical conditions are changing sufficiently rapidly, early in this phase, so that the material is (probably) not in equilibrium. Finally, it is not clear that a given abundance solution, obtained only from ratios of lines, is unique. In an attempt to ameliorate these problems, new optimization methods have been developed to determine nova ejecta abundances (Vanlandingham et al. 1996; Vanlandingham, Starrfield, & Shore 1997, and references therein).
The rate of expansion of the ejecta is the most important difference between novae, classic H ii regions, and planetary nebula shells. To a first approximation, neglecting the effects of the wind from the central star, the ejected nova shell has a fixed mass, and, therefore, its density is uniquely determined by the velocity field imposed at the time of the explosion. The ejection velocities are much higher for novae than for either H ii regions or PNe, and thus, they evolve much faster in time. The ejecta also have inner and outer radii that scale self‐similarly [ΔR/R(t) = constant]. Consequently, the density, which is a power law in radius, scales throughout the ejecta as a power law with time. This implies that the velocity width of a line is an indication of the depth at which it is formed in the expanding shell. Since emission lines are formed only above the photosphere (see above), the mass depth at which the lines are formed is governed by the wavelength dependence of the opacity. For instance, emission lines appear earlier in the infrared because the line opacity is lower in that spectral region than in the optical or UV. The combination of the drop in density, which rapidly reduces the emission measure for the IR lines, and the increase in the ionization implies that changes in the IR occur before the changes observed in other wavelength regions. This is observed (Hayward et al. 1996).
Throughout the outburst, the central star dominates the energy balance of the expanding ejecta. Since the source remains at, or near, constant bolometric luminosity for some period of time (Starrfield 1989; Shore et al. 1993, 1994a, 1994b), the radiation that reaches the (outermost) optically thin parts of the ejecta is due entirely to reprocessing through the underlying material. Initially shielded by the gas that is still optically thick in the absorption lines, the "color" temperature of the source gradually increases as the pseudo‐photosphere moves inward to deeper and hotter layers. The first emission lines to appear in the UV and optical are intercombination lines. This is caused by the large spatial extent of the optically thin material and the decreased collisional de‐excitation of the upper radiatively excited levels. These lines are accompanied by the increasing strength of IR forbidden transitions from species such as [Ne ii] that are formed in the highest velocity portions of the ejecta. Finally, the illuminating radiation becomes hard enough to completely ionize the ejecta (Krautter et al. 1996; Shore, Starrfield, & Sonneborn 1996). At this time, around UV maximum, the combined effects of decreasing density and increasing "color" temperature ionize the low‐ionization atoms that form the dominant source of line opacity and the matter experiences a dramatic increase in FUV and EUV flux. This marks the onset of the nebular stage.
Although the postoutburst geometry of the ejected shell may be poorly known and is obviously complicated, the line profiles provide important information. If the profiles are truly optically thin, each part of the line arises from the same region of the shell regardless of the ionization stage being observed. Contemporaneous multiwavelength observations, at sufficiently high resolution to obtain profile data of order 50–100 km s−1, can thus serve to specify the run of ionization throughout the optically thin parts of the ejecta (see, e.g., Shore et al. 1993). In addition, since the elemental abundances in any knot or region of the expanding matter are constant in time, use can be made of time sequences of the line profiles to remove much of the ambiguity otherwise introduced by the complexities of the geometry.
Reliable element abundance determinations at this time require, in principle, a knowledge of the individual abundances for all ionization stages that are relevant for each element, even though many of these may not have lines that are in accessible portions of the spectrum. Line ratios alone provide only ion ratios. Because of the large velocity and, thereby, density range in nova ejecta, even lines formed from species with similar ionization potentials can be formed by a number of different processes including radiative excitation, collisional excitation, or recombination. These processes can best be accounted for by modeling that includes the large variations in density that characterize nova shells. Modeling of the line profiles have been performed by Hutchings (1972) for HR Del and Shore et al. (1993) for V1974 Cyg 1992, who used a Monte Carlo treatment for the line formation. An important byproduct of this modeling is obtaining a value of the filling factor, which is necessary for the determination of elemental abundances. The modeling shows that nova line profiles can be broadly characterized by two components: diffuse material that dominates the high‐velocity parts of the ejecta and knots, that are found mainly in the inner, slower moving material. The density contrast between these two regions is easily seen in the evolution of line profiles observed with sufficient spectral resolution through the early stages of the outburst and individual knots can be identified in many different ionization stages. As the outburst proceeds, the contrast grows between the knots and the diffuse gas, making the abundance determinations from line profiles progressively easier.
One difficulty with modeling the later stages of the emission‐line evolution is the development of the central star. Although it is possible to follow its evolution in X‐rays (Krautter et al. 1996) and the UV (Shore et al. 1996), this is not as easy in other wavelength regions. The observations of the evolution of both GQ Mus (Shanley et al. 1995) and V1974 Cyg (Krautter et al. 1996) show that the length of the nuclear burning stage is quite variable. ROSAT observations of 55 recent nova outbursts imply, in fact, that the nuclear burning time is typically less than 10 yr (Orio 1998). Once nuclear burning ceases and the illuminating X‐rays stop maintaining the ionization, the ejecta begin to recombine. The ionization of the ejecta initially increases as the X‐ray emission increases, and it then decreases because of the onset of recombination. The continuing expansion, however, also causes a decrease in recombination since the density continues to decline, and the competition between these two effects eventually leads to a freeze‐out of the ionization fractions of the ejecta. The continuing decrease in density, thereafter, causes the emission lines to steadily weaken because of the drop in the emission measure [which varies as R(t)−3], but the relative ion contribution to the abundances remains roughly constant (Krautter et al. 1996; Shore et al. 1996).
The quantities that are involved in the initial determinations of the abundances of nova shells using optical/UV lines are given in Table 4. Line intensities must be corrected for extinction, and a somewhat reliable method to achieve this is to compare lines originating from the same levels, whose intrinsic intensities are therefore known (Draine & Bahcall 1981; Osterbrock 1989; Williams 1994). Because of the paucity of lines, however, alternative methods have been tried, and one normally uses an average value obtained from a variety of methods (Schwarz et al. 1997c; Vanlandingham et al. 1996, 1997, 1998).
The early methods for determining abundances in novae from optical/UV lines came from direct comparisons of line intensities, which are dependent upon relative ion abundances, ne, and Te. Since physical conditions vary widely in the supersonically expanding ejecta, implying that the structures are frozen into the flow and evolve locally depending only on their density, the densities and temperatures must be derived for the regions of gas from which the respective lines are emitted. This is best done for the spatially resolved shells of old novae, and it is not very accurate for the shells of novae observed just after the outburst. Since the relative intensities of the various emission lines of an individual ion depend only on ne and Te, one can derive temperatures and densities from such lines see Seaton 1960; Shaw & Dufour 1995). The stronger optical/UV lines of the heavy‐element ions that can be used for this purpose in novae are given in Table 4. In order to minimize the dependence of derived elemental abundances on ne and Te, lines should be selected that have similar critical densities and Boltzmann factors. Networks of such lines for various ionization levels have been defined by Williams (1995), and in Table 4 we list some of the best transitions meeting the criteria listed above. A compact notation has been used to list the transitions, and IR lines have also been included for completeness. Any emission‐line ratio may be used to deduce a relative abundance for two ions, but the lines given in Table 4 are those for which any dependence upon temperature and density should be small.
There are a number of novae that have displayed outbursts in the last decade for which postoutburst abundances were determined by one or another of the methods described above (Snijders et al. 1987; Williams 1985; Snijders 1990; Saizar et al. 1991, 1992; Andreä et al. 1994). Nevertheless, the discrepancies between different studies of the same nova indicate that they provide only a first step in the analysis of the ejecta. A better way to determine nebular abundances in young novae is to use a photoionization program, such as CLOUDY (Ferland 1996), in combination with an optimization technique. The benefit of such a procedure is that one obtains a global solution for all elements simultaneously, and one can then determine a statistically robust estimate of the errors in the abundances. This is not available from the line ratio method. In addition, since the nebular model provides the density as a function of radius, it becomes easy to determine the total ejected gas mass. Although CLOUDY does not currently treat density inhomogeneities in the gas, neither does the line ratio method.
The optimization methods require an initial guess of the abundances, ejecta radii, filling factor, and covering factor (Austin et al. 1966; Vanlandingham et al. 1996, 1997). In addition, one can assume a density gradient that comes from dynamic modeling of the outburst. These parameters are then used as input for a CLOUDY integration through a model of the ejected shell, resulting in a prediction of the strengths of a large number of emission lines. These predicted line strengths are then compared to the observed line strengths and a value of χ2 is determined, which measures the accuracy of the fit of the model to the observations. At this point a new set of initial parameters is chosen, and another integration of CLOUDY is performed. The iterations continue until a minimum global χ2 is obtained.
Austin et al. (1966) used a simulated annealing method (Metropolis et al. 1953; Press et al. 1992) to choose their iteration parameters, which required a large number of iterations (10,000) to treat V1974 Cyg. This method, however, did not converge for the physical conditions found for V831 Her/1991 (Vanlandingham et al. 1996). We note that Austin et al. (1966) determined the abundances only for He/H, N/H, O/H, Ne/H, and Fe/H. The abundance results listed in their Tables 24 and 25 included values for elements for which no lines were available to constrain the results. A better procedure is to use is the Davidson‐Fletcher‐Powell variable metric algorithm (Press et al. 1992) as provided in the MINUIT program from CERNLIB (James & Roos 1993). MINUIT uses CLOUDY as a subroutine and rapidly drives the value of χ2 to low values in 2000 iterations. It is then possible to vary the input parameters, such as the abundances, and determine the errors associated with each parameter. This has now been done for V838 Her/1991 (Vanlandingham et al. 1996), V693 CrA/1981 (Vanlandingham et al. 1997), and PW Vul/1984 (Schwarz et al. 1997c). Studies of LMC 1990 1 and LMC 1991 are now in progress (Vanlandingham et al. 1998; Schwarz et al. 1998, in preparation).
The results for all of these novae have proved to be quite interesting and are given in Table 2. For example, Schwarz et al. (1997c) have reanalyzed the IUE and optical data for PW Vul and discovered the causes of the discrepancy in the abundances between the studies of Andreä et al. (1994) and Saizar et al. (1991). The abundances of Schwarz et al. are given in Table 2 and fall in between the values of Saizar et al. and Andreä et al., which are also given in Table 2. Briefly, Schwarz et al. found that Andreä et al. underestimated the absolute fluxes of the optical emission lines by 15%, which lead to a factor of 2 increase in their derived abundances. The abundances of Saizar et al. are smaller than those of Schwarz et al. because they used lower values of the electron temperature in deriving their abundances. In another study, Vanlandingham et al. found that the large‐aperture IUE spectra analyzed by Williams et al. (1985) and reanalyzed by Andreä et al. (1994) were all overexposed and, thus, unsuitable for study. Fortunately, small‐aperture spectra were available on the same dates and were not overexposed. Vanlandingham et al. used only line ratios so that the unknown flux calibration of the small‐aperture spectra did not affect the results. Interestingly enough, their results are within the quoted errors of Williams et al. (1985). Williams (1997, private communication) informed Vanlandingham et al. that he was aware that the lines were overexposed, and this was included in the analysis reported in Williams et al. (1985). Nevertheless, Vanlandingham et al. were unable to reproduce the line fluxes published in Williams et al. (1985).
Since such studies are based on emission‐line fluxes, they sample only the gas and not the dust, although some extinction effects may hamper the comparisons if the dust is not uniformly distributed within or across the ejecta. The relative dust‐to‐gas ratio is difficult to determine, and therefore the depletion of refractory elements due to dust condensation is still unknown. In some novae that form optically thick dust shells, the evolution of the emission spectra of these elements suggests that a substantial fraction of condensables such as C and O may precipitate into grains in the months after outburst. The gas‐phase abundances will, therefore, not reflect the actual elemental distribution produced by the outburst and injected into the ISM. Starrfield and his collaborators are now attempting to determine the amount and abundances of the gases that condense into grains by analyzing the same nova in both the optically thick and optically thin phases.
It is often the case that thermal emission from dust is absent from the IR spectra of novae when IR forbidden‐line emission is important. Thus, one can presume that the emission‐line phase refers to an epoch when the condensable elements are still mainly in the gas phase. Nonetheless, since evidence for extensive dust formation occurs in only about one‐third of all novae, the gaseous abundances of a sample of novae should, in fact, characterize the true abundances of typical nova ejecta rather well. A compilation is given in Table 2 of gas‐phase abundances for those classical novae whose UV/optical emission spectra were analyzed in the past 15 yr. In spite of the uncertainties associated with the results for individual objects, certain features are clear and may be taken to be general characteristics of nova outbursts: (1) He is generally enhanced with respect to H in the ejecta; i.e., H is depleted. (2) The CNO nuclei are highly enriched, with N typically being the most abundant of these elements. (3) Approximately 25% of the novae show a strong enrichment of neon. These "neon" novae usually also show enhancements of elements with Z≥10, e.g., Mg, Al, and Si.
The first two of these characteristics derive in part (e.g., H depletion and high N) from the outburst phenomenon, since these features follow naturally from the proton‐capture reactions that occur in the TNR. It has been recently realized, in addition, that the high helium abundance also comes from material that has been left on the star from the previous outburst (Krautter et al. 1996). The high neon abundance in "neon" novae is difficult to produce in normal outbursts on CO WDs and can only result from material mixed up from the cores of ONeMg WDs (Starrfield, Sparks, & Truran 1986; Truran & Livio 1986; Starrfield et al. 1993). Moreover, the high CNO abundance enrichments also demand outward mixing of core matter (Starrfield 1989; Truran 1990; Livio & Truran 1990).
Spectroscopy of the emission lines from ions does not enable isotopic abundances to be determined; the isotope shifts are too small to be detected. Thus, the neon could be 20Ne or 22Ne, and the aluminum could be26Al or 27Al. Any isotopic information must be deduced from theory or, as with 22Na, from γ‐ray observations of novae after outburst. The direct evidence for the gaseous contribution of novae to the element abundances of the ISM is limited to the information in Table 2. Gas of this composition is ejected into the Galaxy by roughly 35 novae yr−1 (Shafter 1997), each of which contributes about 10−4 M⊙ of gas at velocities usually exceeding the escape velocity of the Galaxy (300 km s−1 for a Galactocentric distance of 10 kpc). Thus, the ejecta must interact with a greater mass of ambient gas if they are to be confined to the Galaxy, eventually settling into the ISM.
5.3. IR Fine‐Structure Lines
Many novae, especially those in the ONeMg class, develop IR emission lines from transitions among the fine‐structure levels of the ground states of ions of the heavy elements. Although lines such as [Ne vi] 7.6 μm and [Ne ii] 12.8 μm do occur and can be dominant sources of cooling of the ejecta in some ONeMg novae (see Gehrz et al. 1986, 1994; Hayward et al. 1992), it is more common that higher ionization stages predominate in the IR spectra, e.g., [Mg viii] 3.02 μm and [Si ix] 3.92 μm (Grasdalen & Joyce 1976; Greenhouse et al. 1988, 1990; Woodward et al. 1995). Because of the very low excitation potentials of the IR lines, kTe>12 eV for all such transitions, the line intensities do not depend on Te. Relative strengths of the IR lines yield reliable ion abundances, since they are probably all collisionally dominated, leading to the same dependence upon density. Intercomparison of these lines produces relative abundances of Mg, Na, Al, and Si (see Table 6).
V1974 Cyg/1992 was the first ONeMg novae for which extensive IR spectroscopy of the coronal phase (see Table 6) was available to augment the UV/optical studies. It was the brightest northern hemisphere nova since V1500 Cyg/1975, and IR observers were able to use it to construct a rather complete picture of the temporal development of a neon nova. Strong [Ne ii] emission at 12.8 μm and hydrogen emission dominated the 7–14 μm spectrum 54 days after the outburst, and hydrogen and helium lines were the principal emission features in the near‐IR for the first 100 days (Dinerstein et al. 1993). V1974 Cyg/1992 entered a coronal phase several hundred days after outburst (see Gehrz 1993, and the references therein). By day 270, the temperature of the central engine had increased sufficiently to drive the Ne ii to higher ionization states. A series of observations with SpectroCam‐10 (Hayward et al. 1996) and the HIFOGS IR spectrometer operating on the NASA Kuiper Airborne Observatory (KAO) and at Mount Lemmon, Arizona (Gehrz et al. 1994), showed that by day 270 the 12.8 μm [Ne ii] emission line had decreased sharply, while 7.6 μm emission from [Ne vi] had grown very strong. V1974 Cyg/1992 is still sufficiently bright that IR observers continue to monitor its posteruptive spectral development. These observations include periodic measurements to determine metal abundances using both ground‐based telescopes (Woodward et al. 1997) and the ISO satellite (Salama et al. 1996). In general, the rather large abundances of Ne, Al, and Mg with respect to H and Si tend to confirm the models of TNRs on ONeMg WDs proposed by S. Starrfield, J. Truran, W. Sparks, and their collaborators. The conformance between the observations and the abundance elevations predicted by these models requires that a considerable amount of material be dredged up from the outer layers of the WD at some phase of the TNR.
5.4. Physics of the IR Coronal Phase
IR forbidden lines have been identified in a number of recent ONeMg novae including QU Vul/1984 #2, V827 Her/1987, V2214 Oph/1988, and V1974 Cyg/1992 (Grasdalen & Joyce 1976; Gehrz et al. 1985; Greenhouse et al. 1988, 1990; Benjamin & Dinerstein 1990; Hayward et al. 1992, 1996; Woodward et al. 1995), confirming a theoretical prediction by Ferland & Shields (1978a, 1978b) that such emission could be an important source of cooling in nova shells. The strongest of these lines was 12.8 μm [Ne ii] emission from QU Vul/1984 #2 (Gehrz et al. 1985). Greenhouse et al. (1988, 1990) established the practicality of using these IR "coronal" emission lines for evaluating physical conditions and chemical abundances in the ejecta. While the IR transitions themselves are collisionally excited, the ionization states responsible for the transitions must be largely radiatively excited (Benjamin & Dinerstein 1990; Williams 1990). In some cases, it appears possible that the ionization structure may be augmented by a collisional excitation component (see Greenhouse et al. 1990). Temperature conditions associated with the coronal emission are not fully understood. Radiation temperatures inferred for the photoionization of the coronal states are as high as 5 × 105 to 106 K. However, the absence of certain lines that would be expected to occur suggests kinetic temperatures well below this. Infrared speckle interferometric measurements of QU Vul by Greenhouse et al. (1990) showed that the coronal emission occurs in the expanding ejecta rather than close to the central engine. High‐resolution near‐IR spectroscopy of several other ONeMg novae shows that the velocity widths of coronal lines are likewise consistent with the conclusion that these lines arise in the expanding ejecta (Hayward et al. 1992, 1996; Woodward et al. 1995).
Analyses of the abundances of the coronal species also show that the ejecta of many ONeMg novae are rich in Ne, Mg, Al, and Si. Observations of QU Vul/1984 #2 (Gehrz et al. 1985; Gehrz et al. 1986; Greenhouse et al. 1988, 1990; Gehrz et al. 1995b) and V1974 Cyg/1992 (Hayward et al. 1992; Woodward et al. 1995; Hayward et al. 1996; Salama et al. 1996) have been especially rich sources of information about the gas‐phase abundance anomalies that can be produced by TNRs on ONeMg WDs (see Table 6). The Salama et al. (1996) paper reporting IR spectroscopic data obtained on V1974 Cyg/1992 with the European Space Agency (ESA) Infrared Space Observatory (ISO) satellite presages the exceptionally important role that future space observatories will have in using IR forbidden‐line emission to evaluate elemental abundances in nova ejecta. With satellites, one can observe line emission that is inaccessible from the ground from complete isoelectronic sequences of important elements thus permitting the calculation of self‐consistent abundances.
As we have described above, the nucleosynthesis associated with such nova explosions can produce significant quantities of the radioactive isotopes22Na (2.7 yr) and 26Al (τ1/2 = 7.2 × 105 yr), that positron decay to 22Ne and 26Mg. These materials could be trapped in grains during the dust formation phase of novae, which is observed to occur on a timescale less than 1 yr. Grain inclusions with significant overabundances of 22Ne and26Mg have been discovered in solar system meteorites. The inference is that these meteoritic inclusions could have been produced by the condensation of grain materials in the immediate vicinity of sources of explosive nucleosynthesis, such as novae or supernovae (see Clayton 1982; Truran 1985, 1990; Gehrz 1988; Weiss & Truran 1990). Infrared observations have confirmed that modest amounts of dust have condensed in the ejecta of several ONeMg novae. QU Vul/1984 #2 produced silicate dust (Gehrz et al. 1986), and carbon dust formed in the circumstellar winds of V827 Her/1987 (Gehrz 1988) and Nova Aql/1995 (Mason et al. 1996).
6. INFRARED OBSERVATIONS OF DUST FORMED FROM HEAVY NUCLIDES
Although transient dust formation events are believed to have been observed in the Wolf‐Rayet star HD 193793 (Hackwell, Gehrz, & Grasdalen 1979), SN 1987a (Gehrz & Ney 1987, 1990), and the post‐AGB star FG Sge (Woodward et al. 1993), the most convincing cases for transient circumstellar dust formation are those based upon IR observations of the temporal development of CO nova systems (Bode 1988a, 1988b; Bode & Evans 1989; Gehrz 1988, 1990, 1993, 1995; Starrfield 1988, 1989, 1990). The IR characteristics of CO novae progress in several identifiable stages (see Gehrz 1988). The initial eruption results from a TNR on the surface of the CO WD. The hot gas expelled in the explosion is initially seen as an expanding photosphere, or "fireball." IR free‐free and line emission are observed when the fireball becomes optically thin. A dust condensation phase, characterized by declining visual light and rising IR emission, occurs in many novae within 50–200 days following the eruption. The IR emission continues to rise as the grains grow to a maximum radius of 0.2–0.55 μm within a few hundred days after their condensation and then falls as the mature grains are dispersed by the outflow into the ISM (Gehrz et al. 1980a, 1980b). Thus, nova grains initially grow much larger than interstellar grains, which typically have radii in the range from 0.01 to 0.12 μm (see Greenberg 1989). However, the rate of decline of the IR radiation and the relatively slow cooling rate of the grains in the outflow suggest that the grains begin to decrease in radius again shortly after having grown to their maximum size. The observations are consistent with the hypothesis that the nova grains could be processed to interstellar grain sizes by evaporation or sputtering before they eventually reach the ISM. About 10−8–10−6 M⊙ of dust forms in each episode. Physical parameters of the dust shell are defined in Table 3, and Table 5 gives measured values for a number of novae.
6.1. Mineral Composition of the Grains and the Nucleation Process
Most dust‐producing CO novae condense dust that emits a smooth 1–25 μm blackbody (possibly graybody in some cases) continuum that is devoid of emission features. Both carbon and iron grains can produce this spectral signature (see Gilman 1974a, 1974b; Draine 1985). Because a similar continuum is characteristic of the circumstellar emission from carbon‐rich stars (see, e.g., Gehrz & Hackwell 1976), it has generally been assumed that this continuum in classical nova systems is produced by amorphous carbon grains (see Gehrz 1988). The genesis of the argument that smooth IR continua result from circumstellar carbon dust in carbon stars is a set of equilibrium condensation calculations (see Gaustad 1963; Hackwell 1971, 1972) showing that carbon grains condense if C > O, as would be expected for the ejecta of carbon‐rich stars, and that silicate grains condense if O > C, as would be expected for oxygen‐rich giants. In this scenario, C and O combine to form CO until all the less abundant condensable is consumed, leaving the remainder of the more abundant condensable as a primary grain constituent. The formation of CO just prior to the dust condensation phase has been well documented by IR spectroscopy of several CO novae including NQ Vul/1976 (see Gehrz 1988) and V705 Cas/1993 (Evans et al. 1996). A fundamental problem with the carbon grain hypothesis for novae is that it may be unlikely that C > O can occur in nova ejecta that result from TNRs on CO and ONeMg WDs; theoretical models generally predict that C < O for TNRs on both CO and ONeMg WDs. Moreover, optical and UV spectral studies of novae (see our Table 2) indicate that generally O > C in the gas‐phase ejecta (Truran 1990). If this is the case, the formation of carbon grains seems improbable, unless the condensation of carbon proceeds in a rather different manner than predicted by the equilibrium calculation.
An alternative explanation for the continuum dust emission in novae is that the grains are iron. One can use the opacities and Planck mean cross sections given by Gilman (1974a, 1974b) to estimate the mass of iron dust that would be required to produce the observed visual extinction in a typical optically thick CO nova shell. As an example, we consider NQ Vul/1976, a CO nova that formed a shell with a visual optical depth of τv ≈ 4.6 about 80 days after the eruption; the shell involved 10−4 M⊙ of gas expanding at 750 km s−1. In this case, the observational parameters require a dust mass of 10−6 M⊙ of iron or 1.4 × 10-7 M⊙ of carbon, leading to gas‐to‐dust ratios of 100 and 700 for iron and carbon, respectively. The gas‐to‐dust ratios for iron and carbon in solar material are 670 and 250, respectively. We conclude that NQ Vul's ejecta would have had to be enhanced in iron by at least a factor of 7 in a gas of otherwise solar composition for the grains to have been composed entirely of iron. The ejecta of one dust‐forming nova, V1370 Aql/1982, may have been enhanced in iron by a factor of 4 (see Table 2), although there is no obvious way to account for such an iron enrichment in the context of the standard TNR model. Presuming that the dust is carbon, no enhancement of carbon is implied by the visual extinction in NQ Vul/1976, but at least one dust‐forming novae (LW Ser/1978) would require a carbon enhancement of a factor of more than 5 over solar abundance.
Although most novae produce carbon (or iron?) dust, recent observations suggest that CO nova explosions produce every other type of astrophysical grain, including silicates, silicon carbide (SiC), and hydrocarbons (see Gehrz et al. 1984, 1992, 1995a, 1995b; Gehrz 1988, 1990, 1993; Greenhouse et al. 1988, 1990; Hyland & MacGregor 1989; Mason et al. 1997). In fact, the formation of more than one type of grain in the ejecta from a single outburst has now been recorded for at least four novae. V1370 Aql/1982 produced both carbon and either pure SiC or a combination of SiC and silicates that caused a single broad 7–14 μm emission feature (Gehrz et al. 1984). V842 Cen/1986 produced carbon, hydrocarbon, and silicate dust (Hyland & MacGregor 1989; Gehrz 1993). QV Vul/1987 and V705 Cas/1993 produced carbon, silicate grains, and hydrocarbon dust (Gehrz et al. 1992, 1995a; Mason et al. 1997).
A significant question is how grains can nucleate and grow in the hard radiation field of the central engine. It is generally suspected that the grains grow by condensation on seed nuclei that form in knots (or "clumps") in the ejecta where they are shielded from the extreme‐UV radiation field emitted by the central engine (see Rawlings 1988; Martin 1989; Bode & Evans 1989). The visual light curve of V842 Cen/1986 provided some interesting circumstantial evidence for the presence of clumps in the ejecta. The very deep and short‐lived minimum during the transition phase could have been caused by a dense dust knot that obscured the line of sight momentarily (Gehrz 1990).
The physical processes leading to the formation of several types of grains in a single eruption are not understood. Hard radiation from the nova remnant may provide the UV radiation that is believed to be important in creating hydrocarbons from amorphous carbon (see Allamandola 1984; Allamandola, Tielens, & Barker 1987). Thus, it might be expected that hydrocarbon signatures would be observed frequently in CO novae that make carbon dust. While the source of hydrocarbon emission in some astrophysical sources may be polycyclic aromatic hydrocarbons (PAHs) as proposed by Allamandola (1984) and Allamandola et al. (1987), Evans & Rawlings (1994) have argued persuasively that the physical conditions in nova ejecta favor the interpretation that the emission features are due to hydrogenated amorphous carbon (HAC) grains.
Calculations of high‐temperature equilibrium condensation of molecules in carbon‐rich gases using available thermochemical data by Sharp & Wasserburg (1995) show that it is plausible that both carbon and SiC grains can condense in carbon‐rich ejecta. On the other hand, it is difficult to understand how both silicate grains and carbon grains can form the same object if silicate grain condensation requires an environment where C < O and carbon grain condensation requires an environment with C > O (see Hackwell 1971, 1972; Sharp & Wasserburg 1995). One possibility is that there are significant abundance, pressure, temperature, and density gradients within the ejecta. In particular, the novae that form multiple grain types may require a rather complex temporal evolution of these gradients. For example, once the constant luminosity phase has ended and the luminosity of the central engine begins to decline, the dust condensation zone may sweep rapidly down into the inner regions of the shell where densities are higher and where chemical abundances may have been altered during the postoutburst common envelope‐burning phase. There is also evidence that the polar plumes and equatorial rings believed to be produced in the explosion as a result of rotation may have different properties (see Gallagher & Starrfield 1978; Solf 1983).
Finally, there is the question of whether ONeMg novae are implicated in the production of solar system meteorite inclusions carrying the 22Ne (neon‐E) and 26Mg anomalies. At least one of these novae, QU Vul/1984 #2, has been observed to have both strong [12.8] μm [Ne ii] emission (Gehrz et al. 1985) and a silicate dust formation phase (Gehrz et al. 1986). V1370 Aql/1982, which showed an enormous overabundance of neon, condensed an optically thick shell of carbon and SiC/silicate dust (Gehrz et al. 1984), and carbon (iron?) dust condensed in the ejecta of neon‐ and sulfur‐rich V838 Her/1991 (Woodward et al. 1992; Vanlandingham 1997). In all of these cases, it seems possible that condensables such as 22Ne, 22Na, 26Mg, and 26Al could be incorporated into the grain structures that grow in the ejecta under the right physical conditions. It is noteworthy that some carbon (graphite and diamond), silicate related oxide (26Mg enriched Al2O3), and SiC grains identified in meteorite inclusions are believed to be preserved presolar interstellar dust grains (Anders & Zinner 1993; Ott 1993; Hutcheon et al. 1994). ONeMg novae are predicted to be rich sources of all of the constituents of these molecules.
6.2. Determining Abundances of Metals from Observations of Dust
IR measurements of classical novae have proven useful for definitively quantifying many astrophysical parameters that describe the outburst and subsequent development of the expanding ejecta. Table 3 summarizes the properties of novae that can be directly measured by IR observations and the astrophysical parameters that can be calculated by applying these parameters to a uniformly expanding, constant velocity, ejected shell. The relationships given in the third column are gleaned from many studies of the IR temporal development of novae that have been conducted during the past 20 years (see the discussions presented by Gallagher & Ney 1976; Ennis et al. 1977; Ney & Hatfield 1978; Bode & Evans 1989; Gehrz et al. 1980a, 1980b, 1985; Gehrz & Ney 1987, 1990; Gehrz 1988, 1990, 1993; Hayward et al. 1992). Optically thick fireballs of all novae and optically thick dust shells formed by CO novae produce blackbody spectral energy distributions (SEDs) in the IR. The apparent luminosity of the SED is particularly straight forward to estimate in these cases, since the integrated flux F under a blackbody energy distribution with temperature T is measured by the quantity (λFλ)max = (νFν)max, where F = 1.36(λFλ)max (Gehrz & Ney 1992). Blackbody angular radii can be combined with Doppler expansion velocities inferred from optical/IR spectra to yield the distance to a nova and therefore its absolute luminosity. In cases where this method has been successfully applied, the outburst luminosity is always seen to be greater than or equal to the Eddington luminosity (see eq.[3]).
As discussed above, the dust that forms in nova ejecta is mainly composed of carbon, although small amounts of silicon carbide (SiC), oxygen‐rich silicate minerals based on SiO2 molecules (such as olivine, enstatite, and pyroxene), and hydrocarbons (PAHs) have been observed to form in the ejecta of a small number of novae (Gehrz 1988, 1990, 1993; Gehrz et al. 1992; Mason et al. 1997). The strengths of dust emission features and dust continuum emission can be used to determine abundances of the condensable elements in nova ejecta. Table 5 summarizes our knowledge of outburst and shell parameters for 25 recent novae calculated from optical/IR observations using the relationships given in Table 3. The mass of gas in the ejecta can be measured using the energy distribution, as determined by IR techniques. As the expanding photosphere becomes optically thin, the energy distribution deviates slightly from that of a blackbody, but the ejecta are still hot enough that the Thomson scattering opacity κT (cm2 g−1) dominates radiative transfer in the shell. In this case, the shell mass is given by M(g) = πR2κ-1T, where Vo (cm s−1) is the outflow velocity and R(cm) = Vo t is the shell radius at time t seconds after the outburst. Later, when the ejecta cool sufficiently that thermal Bremsstrahlung dominates the shell opacity, the gas mass of a shell of depth l pc and electron density ne (cm−3) can be determined through the relationships n2eλ2c l = 1018 and M(g) =9.7 × 1018 R2 l ne H, where λc(μm) is the "cutoff" wavelength at which free‐free self‐absorption becomes important and H =1.66 × 10-24 g is the mass of the nucleon. The mass of dust can be inferred from the dust shell opacity required to produce the observed visual extinction for novae that produce optically thick shells. Opacities for carbon, SiC, silicates, and iron are given by Gilman (1974a, 1974b) and Draine (1985). The gas‐to‐dust ratios calculated in this way imply overabundances of carbon and silicate materials in a few cases.
V705 Cas/1993 presented a classic example of dust formation in CO novae and provides a paradigm for applying the relationships given in Table 3 (Gehrz 1995; Gehrz et al. 1995a; Mason et al. 1997). The visual extinction event (transition) caused by the formation of its dust shell was the largest observed since the probable dust formation episode in DQ Her/1934 more than 60 years ago. The visual extinction and IR dust emission both reached a maximum around day 106. We can apply the parameters described in Table 3 to determine the physical characteristics of the dust that formed in the ejecta of V705 Cas/1993. Correction for interstellar visual extinction leads to the conclusion that the dust shell of V705 Cas/1993 reradiated about 70% of the outburst luminosity. Presuming from the deep visual transition that the shell was optically thick at visual wavelengths, the relationships in Table 3 imply a luminosity of Lo = LIR for the central engine on day 106. Infrared spectroscopy by Woodward & Greenhouse (1993) gives an expansion velocity for the principal ejecta of Vo = 840 km s−1. On day 106, the dust shell had an angular radius of r = 22 milliarcsec (mas), a temperature of TBB = 690 K, and a peak apparent flux of (λFλ)max = 1.1 × 10-14 W cm−2. The resulting distance of 2.33 kpc leads to a luminosity of Lo = 2.5 × 104 L⊙, which is at the Eddington luminosity for a 0.7 M⊙ WD. This is entirely consistent with the picture that the most extreme CO novae occur on rather low‐mass WDs. The dust grain radius required to produce the observed IR maximum luminosity on day 106 is agr = 0.55 μm. Independent confirmation that large grains formed in the ejecta of V705 Cas/1993 comes from an analysis by Shore et al. (1994) of the UV circumstellar dust extinction using IUE spectra taken during the dust formation phase. They found that the grains had already grown to radii of about 0.2 μm by 76.5 days after the outburst. Gehrz et al. (1980a, 1980b) also found evidence for large grains in the ejecta of LW Ser/1978 and V1668 Cyg/1968. We note that these grains are significantly larger than the grains that cause the general interstellar extinction but are similar in size to the small grains that make up interplanetary dust particles (IDPs; see Brownlee 1987). The total carbon dust mass implied by the IR luminosity on day 106 is Md = 6 × 10-7 M⊙, and the fact that the fireball had become optically thin by day 7.5 puts an upper limit on the gas mass at that time of Mgas<1.3 × 10-5 M⊙. Thus, the gas‐to‐dust mass ratio is ∼22 to 1, and given the solar value of 275 for the mass ratio of hydrogen to carbon, we conclude that carbon is at least a factor of 12.5 overabundant in V705 Cas/1993. Hauschildt et al. (1994b) report that CNO enhancements of 100 or more are implied by IUE UV spectra taken before the dust formation episode.
7. SUMMARY AND CONCLUSIONS
Material processed by explosive hydrogen‐burning nucleosynthesis is intermittently injected into the ISM by classical novae explosions caused by TNRs on the surfaces of WDs accreting matter in close binary systems. We have described (§2) the physics of the TNR and shown that the mass and chemical composition of the ejecta depend strongly upon the mass of the WD (see Tables 1 and 2). Infrared observations are shown to be important for determining the primary physical parameters that describe the TNR and subsequent temporal evolution of the ejecta. Table 3 summarizes the theory that can be used to deduce nova physics from the IR data, and Tables 5 and 6 give values measured for these physical parameters by IR observations of 25 recent novae. We have reviewed in §2 theoretical studies that suggest that Li, CNO, and Ne, Na, Mg, Al, S, Si could be enhanced by substantial factors over solar abundances in the ejecta expelled by TNRs on CO and ONeMg WDs. Although novae probably have processed less than ∼0.3% of the interstellar matter in the Galaxy, both theoretical and observational evidence suggests that they may be important sources of the nuclides 7Li, 15N, and 17O, as well as of the radioactive isotopes 22Na and 26Al.
The implications of the theoretical studies for enrichment of the ISM by nova ejecta on both local and Galactic scales have been evaluated in §4, where we conclude that many of the heavy isotope anomalies attributable to novae are most likely produced by the approximately 25%–33% of novae that occur in systems containing massive (M ≳ 1.2 M⊙) ONeMg WDs. We evaluate the degree to which classical novae participate in the production of Galactic 7Li and to the 22Na and26Al chemical anomalies. The latter nuclides are astrophysically important in that they may have been involved in the production of the 22Ne (Ne‐E) and26Mg enrichments identified in meteoritic inclusions whose composition may be representative of the contents of the primordial solar nebula. These inclusions may be partially composed of dust condensed in nova outbursts. Diffuse Galactic γ‐ray fluxes are shown to provide particularly important clues to and constraints upon the22Na, 26Al, and 7Be yields from novae. We emphasize that novae are expected to form substantial amounts of7Be (τ1/2 = 53 days) and22Na (τ1/2 = 2.7 yr), the lifetimes of which are comparable to those of novae in outburst. These decays yield potentially detectable levels of γ‐ray emission, for relatively nearby objects. That no detections of the above γ‐ray sources have been obtained with CGRO instruments from recent novae is a regrettable consequence of the fact that the expected fluxes are at the limit of detectability with CGRO, for novae at distances of order ∼1 kpc.
We may hope to be able to improve upon this situation with the use of future instruments such as the International Gamma Ray Astrophysics Laboratory (INTEGRAL). A detection of a nova in outburst would provide an important confirmation of the essential features of the current paradigm for classical nova outbursts and serve to impose critical constraints on the role of convection in the earliest stages of the TNRs. Methods for obtaining observationally constrained gas‐phase elemental abundances from UV, optical, and IR emission‐line spectra of classical novae for a number of classical novae have been reviewed in §5, and the results are summarized in Tables 2, 4, 5, and 6. Special attention is given to recent studies showing that the ejecta in some novae can be strongly cooled by near‐ and mid‐IR radiation from forbidden‐line radiation from highly ionized ("coronal") atomic states. Table 2 presents the present state of knowledge of the mass fractions in the ejecta of recent novae based on UV/optical spectroscopy. These are given in the form of mass fractions of the elements hydrogen (H = X), helium (He = Y), metals [nuclei heavier than He ("metals") = Z], carbon (C), nitrogen (N), oxygen (O), neon (Ne), and metals heavier than neon including those as heavy as iron (Na–Fe). For purposes of comparison, we note that solar system matter is characterized by a helium‐to‐hydrogen ratio by number He/H = 0.08 and a heavy‐element mass fraction Z solar = 0.019 (see Cameron 1982, 1993; Grevesse & Anders 1989). Table 6 gives new abundance data available through analysis of IR forbidden emission lines. It is apparent that all classical novae with relatively reliable abundance determinations show enrichments relative to solar composition in helium and/or heavy elements. This statement is secure even when uncertainties in the abundance determinations are taken into account. We conclude that envelope enrichment in classical novae is a very general phenomenon. Truran & Livio (1986) argued that it is extremely unlikely that the source of these enrichments is either the mass transfer from the secondary star or nuclear transformations accompanying the outburst, and they concluded that the abundance patterns suggest that some fraction of the core matter is dredged up from the underlying WD. Given the presence of large concentrations of neon and heavier elements in the ejecta of several recent novae (e.g., V693 CrA/1981 and V1370 Aql/1982), it is interesting to note that this conclusion has the consequence that massive ONeMg WDs (as opposed to CO WDs) occur quite frequently in classical nova systems. One important problem that remains to be satisfactorily resolved is the identification of the mechanism that is responsible for the mixing between the accreted envelope and the WD core (see Livio & Truran 1990; Sparks 1995). Four possible mechanisms have been suggested: (1) diffusion induced convection, (2) shear mixing, (3) convective overshoot induced flame propagation, and (4) convection induced shear mixing. Livio & Truran (1990) discuss each of these mechanisms, pointing out their strengths and weaknesses, and attempt to identify critical observations that will help to determine which mixing mechanism is most likely to dominate.
We have described in §6 how IR observations of novae reveal dust formation in most CO novae and in some ONeMg novae. Differences in the IR temporal development scenarios of CO novae and ONeMg novae (neon novae) are compared. Novae produce only about 0.3% of the Galactic "stardust" (dust condensed in stellar outflows), but IR observations show that it may be some of the most interesting dust. Novae appear capable of producing astrophysical dust in situ of virtually every known chemical and mineral composition, and it appears possible that neon novae may lead to the formation of dust grains that carry the Ne‐E and 26Mg anomalies. Finally, we have shown that the IR spectral energy distributions and visual extinction characteristics of the dust shell can be used to derive the abundances of the dust constituents. As in the case of the gas‐phase condensables in the ejecta, the abundances of the metals condensed into solids appear in some cases to elevated well above solar abundance.
This paper was inspired by our attendance at the Abano Terme Conference on Cataclysmic Variables held in Abano Terme, Italy, in 1994 June. R. D. G. is grateful to G. J. Wasserburg for a stimulating discussion about the physical conditions required to explain dust formation scenarios in novae that produce both carbon‐ and oxygen‐rich grains. C. Mason provided last‐minute information about the IR determinations of abundances in V705 Cas/1993 and Nova Aql/1995. S. Shore, P. Hauschildt, G. Schwarz, J. Aufdenberg, and K. Vanlandingham provided critical comments and material for §5. J. Kingdon kindly gave Table 2 a careful reading to ensure its accuracy. Finally, we are grateful to M. A. Greenhouse, T. J. Jones, C. Mason, and C. E. Woodward for reading the manuscript critically as referees. Their suggestions for revisions substantially improved the final product. R. D. G. is supported by NASA, the United States Air Force, the National Science Foundation, and the University of Minnesota Graduate School. J. W. T. and S. G. S. acknowledge support from the National Science Foundation and NASA.